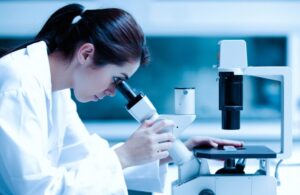
[Adobe Stock]
In molecular biology, small interfering RNAs (siRNAs) have emerged as pivotal tools for regulating gene expression through RNA interference (RNAi). These double-stranded RNA molecules, consisting of 19-23 base pairs, play a crucial role in research and drug development by inducing the degradation of target mRNA and inhibiting gene expression.
This article explores the complex world of siRNA drugs, highlighting their status in the market and their expanding role in clinical research. It explores the early in vivo pharmacokinetic (PK) studies of siRNAs, underscoring the necessity for diverse bioanalytical platforms. This diversity is essential due to the complex behavior of siRNAs in various biological environments. The article examines critical aspects of siRNA analysis, including exposure levels, tissue distribution, the concentration of RNA-induced silencing complexes, and the effectiveness in degrading target mRNA and inhibiting protein synthesis.
By exploring various bioanalytical solutions used in early in vivo PK studies, we aim to provide reliable data and decision-making frameworks, thus accelerating the nonclinical research of siRNA drugs.
Integrated bioanalysis of siRNAs
In siRNAs, the strand that is complementary to the target gene mRNA is the antisense strand (AS), while the other strand is the sense strand (SS). As shown in Figure 1, siRNAs enter cells through endocytosis followed by binding with protein factors, such as Argonaute (Ago), forming RNA-induced silencing complexes (RISCs). The AS is retained in the RISC complex, while the SS is cleaved and degraded. siRNAs recognize target gene mRNA through base pairing. Subsequently, AGO uses its endonuclease activity to cleave the target gene RNA, and the resulting RNA fragments are easily degraded by 5′ or 3′ exonucleases and thus silence the target gene at the post-transcriptional level.
As a type of drug with a novel mechanism of action, siRNAs have broad prospects in treating rare diseases, cancer, metabolic disorders, and other diseases. With the approval of the first siRNA drug in 2018, the development of siRNA drugs is constantly on the rise. As of 2023, more than 200 investigational siRNA drugs are being developed worldwide.
Nonclinical early in vivo PK studies of siRNAs serve as a vital part of the drug development process, investigating the absorption and distribution of siRNAs in organisms and establishing pharmacodynamics (PD) and pharmacokinetics (PK) models. They include evaluations of siRNA plasma PK, tissue PK, RISC PK, target gene mRNA’s degradation efficiency, and target protein’s inhibition efficiency. The bioanalysis of siRNAs contains analysis of siRNAs, RISCs, gene expression, and protein levels. Therefore, multiple analysis platforms are needed to support the quantitative detection of different analytes.
-
Figure 1. siRNA-mediated gene silencing mechanism1
siRNA concentration in plasma/tissue
Most siRNAs are administered via subcutaneous (SC) or intravenous (IV) injection, with a short plasma half-life and reaching maximum concentration within 0.25-2 hours, followed by rapid clearance.2
In early nonclinical in vivo studies, siRNA-related metabolism studies are often lacking. Therefore, plasma siRNA concentrations can be quantitatively determined using liquid chromatography–triple quadrupole mass spectrometry (LC-MS/MS) to avoid the interference of metabolites on the detection of siRNA concentrations. Under denaturing conditions, the double-stranded structure of siRNAs denatures into a sense and antisense strand, which can be detected simultaneously by mass spectrometry with the detection sensitivity of 5–10 ng/mL in plasma.
For siRNA coupled with different delivery systems, suitable bioanalytical methods should be selected based on their structures, such as hybrid-based liquid-phase fluorescence detection (LC-FL), ligand binding assay (LBA), and quantitative polymerase chain reaction (qPCR) to meet quantitative requirements.
Tissue distribution of siRNAs
siRNAs are mainly distributed in a limited number of tissues, including the liver, kidney, renal cortex, and lymph nodes, with a high exposure and a long half-life in the liver.2 There is usually a lag of approximately 7-14 days between the plasma peak concentration and the maximum effect of the siRNA drug, and the pharmacokinetics in plasma and the pharmacodynamics in target tissues do not coincide.3 Therefore, studying siRNA’s tissue distribution and tissue PK is critical for nonclinical early in vivo PK studies.
The concentration of siRNAs in a collected tissue can be quantified using a similar LC-MS/MS method to the analysis method used for plasma samples. Different homogenization schemes must be used for different types of tissues in tissue homogenates. In addition, imaging methods can be used to study the tissue distribution of labeled siRNAs, such as fluorescence imaging of siRNAs labeled with the fluorophore, Cy5 or Cy7. Imaging methods avoid bleeding and cross-contamination during organ harvesting and preserve the structural information of the tissue but have limited spatial resolution and lower detection sensitivity than LC-MS/MS methods.
Intracellular RISC concentration
After entering the body, siRNA must first bind with intracellular Ago and other protein factors to form RISC. The AS of the double-stranded siRNA is retained, and the SS is degraded rapidly. Subsequently, the AS in the RISC binds with the target mRNA to induce mRNA degradation and thus inhibit protein synthesis. This process may take several hours or even days to peak, resulting in a lag in drug efficacy. Therefore, the concentration of the RISC-siRNA complex is closely related to the drug efficacy of the siRNA.
Due to the extremely low content of the RISC-siRNA complex, which may be below 1 ng/g, a more sensitive analysis method is needed for quantitative detection. RISCs can be detected by RNA immunoprecipitation coupled with stem-loop reverse transcription-quantitative polymerase chain reaction (RT-qPCR). This method is a derivative of the stem-loop RT-qPCR method to detect siRNAs in the RISC specifically. The method is divided into three steps: First, magnetic beads bound with protein A/G are conjugated to an anti-Ago2 antibody, then those conjugates capture the Ago2 and RNA complex. After washing and elution, the siRNA bound to Ago2 is obtained and detected by stem-loop RT-qPCR.4 The sensitivity of this method can reach up to 0.25–1 ng/g.
Target gene mRNA degradation efficiency
The mechanism of action of siRNAs is to induce the degradation of target gene mRNA. Therefore, the level of mRNA degradation directly demonstrates the silencing efficiency of siRNA. The relative expression level of target gene mRNAs was examined using the RT-qPCR method with the stable expression of housekeeping genes as a reference to detect the degradation efficiency of target mRNA.
The study process consists of three steps:
- First, total RNA is extracted from the tissue.
- Then, complementary DNA is synthesized using reverse transcriptase.
- Finally, specific primers and reagents are added to amplify and detect PCR.
Two common detection methods are the SYBR Green dye and TaqMan probe methods. SYBR Green is a nucleic-acid-binding dye with weak fluorescence in the free state. When embedded in double-stranded molecules, the fluorescence is greatly enhanced, and this characteristic can be used for quantitative detection. This method has good universality, high sensitivity, economy, and convenience, but the specificity is relatively poor, which may result in non-specific amplification and affect quantification accuracy.
The principle of the TaqMan probe method is that during primer extension, the probe is hydrolyzed, releasing fluorescent groups and thus generating fluorescence for detection. Since the probe and the target sequence are specifically bound, this method has strong specificity, good repeatability, and multiple qPCR analysis can be carried out in a single reaction, which reduces sampling error. The disadvantage of this method is that the probe needs to be specifically designed for each target gene, resulting in poor universality and higher cost.
Inhibition efficiency of target proteins
siRNAs induce the degradation of target gene mRNA, thus affecting the expression of the target protein. However, protein levels are affected by protein expression, stability, half-life, and other regulatory factors. Therefore, the degree of the decrease in the target protein level may not necessarily be proportional to the decrease in the mRNA level. Target protein levels can be detected by enzyme-linked immunosorbent assay (ELISA), Western blotting, or LC-MS methods. When using ELISA to detect target proteins, key reagent availability must be considered. If these reagents are difficult to obtain, Western blotting or LC-MS methods can be used for detection.
A final word
Studying siRNAs in nonclinical early in vivo PK studies presents multifaceted and intricate challenges. siRNA’s unique mechanism of action as gene expression regulators through RNAi offers significant potential in treating a variety of diseases, including rare diseases, cancer, and metabolic disorders. In addition, the degradation efficiency of target gene mRNA and the subsequent inhibition of protein synthesis are critical factors in evaluating the effectiveness of siRNA drugs. The growing number of investigational siRNA drugs—more than 200 in development as of 2023—underscores the importance of comprehensive bioanalytical strategies in their evaluation.
The article demonstrates that the effective bioanalysis of siRNAs requires a multi-pronged approach, incorporating advanced techniques to quantitatively detect siRNAs, RISCs, gene expression levels, and protein concentrations. This integrated strategy is crucial for advancing our understanding of siRNA behavior in biological systems and for refining the development of siRNA-based therapeutics.
Case study: Integrated bioanalysis of GaINAc-conjugated siRNAs
siRNA-X is an siRNA conjugated with N-acetylgalactosamine (GalNAc) with an asymmetrical RNA/dTdT overhang at the 3′ end of the AS. It targets the C5 complement pathway and treats complement-mediated diseases by inhibiting the hepatic production of C5 complement proteins.
We carried out a PK study in mice according to the conventional early in vivo PK protocol of siRNAs. Plasma, serum, and liver samples were collected and analyzed at multiple points after a single administration by subcutaneous injection. The specific study design is shown in Figure 2: three dose groups of low, medium, and high were set, and the mice were tranquilized to collect biological samples at 1 and 6 h and on days 1, 2, 3, 7, 14, and 21 after a single dose.
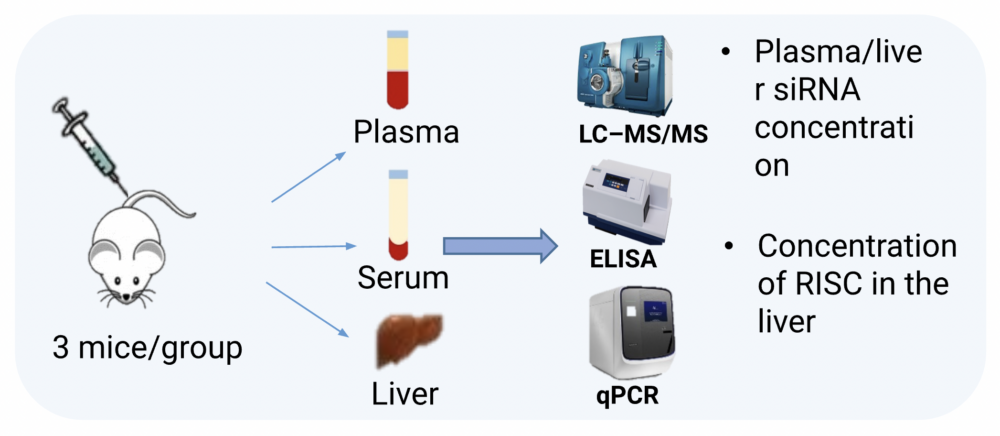
Figure 2. siRNA-X mouse PK study design and bioanalytical solutions
LC-MS/MS, liquid chromatography-triple quadrupole mass spectrometry; ELISA, enzyme-linked immunosorbent assay; qPCR, quantitative polymerase chain reaction; siRNA, small interfering RNAs; RISC, RNA-induced silencing complex
In this study, three different bioanalytical platforms and four bioanalytical methods were used to analyze the samples:
- The plasma and liver concentrations of the siRNA were quantified using LC–MS/MS (Figure 3).
- Levels of C5 complement protein in serum were detected using ELISA (Figure 4).
- The RISC concentration in the liver was determined using RNA immunoprecipitation combined with stem-loop RT-qPCR.
- Target gene expression levels were determined in the liver using stem-loop RT-qPCR.
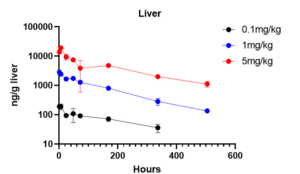
Figure 3. Determination of the siRNA-X concentration in the livers of mice in different dosage groups by LC-MS/MS

Figure 4. Determination of C5 complement protein levels in serum using enzyme-linked immunosorbent assay (ELISA)
By detecting the RISC concentration in liver tissue and comparing it with the inhibition rate of the target protein or the degradation rate of the target mRNA (Figures 5 & 6), the RISC concentration, rather than the drug concentration in free plasma, is more closely related to the gene silencing effect in target.
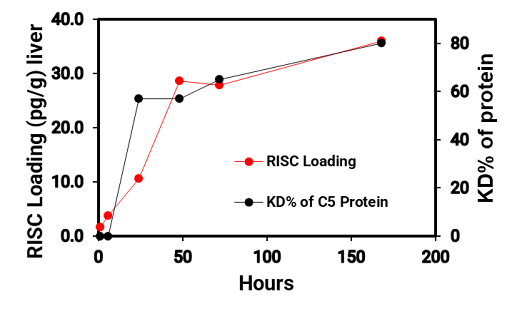
Figure 5. RISC concentration in mouse liver vs. C5 complement protein inhibition rate
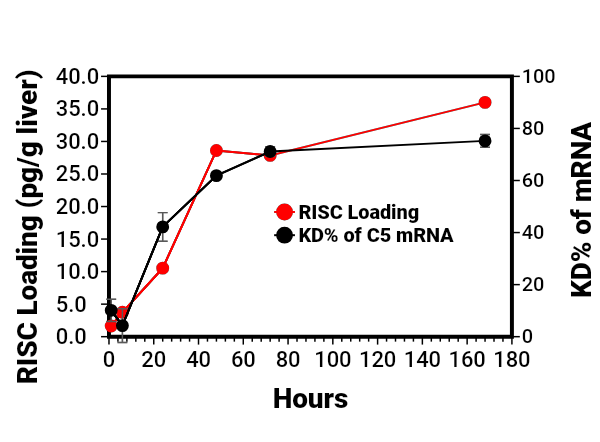
Figure 6. RISC concentration in mouse liver vs. target gene mRNA degradation efficiency
WuXi AppTec’s non-GLP bioanalytical team used five bioanalytical platforms in this study: LC-MS/MS, liquid chromatography–high-resolution mass spectrometry (LC-HRMS), hybrid-based liquid chromatography–fluorescence detection (LC-FL), ligand binding assays (LBA), and qPCR.
These five platforms support oligonucleotide bioanalysis from early drug screening to IND filing, providing diversified bioanalytical solutions. With our extensive experience in oligonucleotide bioanalytical method development, we can deliver high-quality in vitro and in vivo data delivery to accelerate the drug development process.
Guided by the principles of extensive technical expertise and exceptional analysis, we focus on building new analytical capabilities. We continue to build a leading non-GLP bioanalytical service with cross-regional collaboration, scale-up, full process integration, and one-stop service advantages.
References:
- Halib, N.; Pavan, N.; Trombetta, C.; Dapas, B.; Farra, R.; Scaggiante, B.; Grassi, M.; Grassi, G. An Overview of siRNA Delivery Strategies for Urological Cancers. Pharmaceutics 2022, 14, 718.
- Lade, J.M., Thayer, M., Doherty, D., Basiri, B., Xie, F. and Rock, B.M. (2018), Pharmacokinetics, Metabolism, and Biodistribution of a Liver-Targeted siRNA Therapeutic in Preclinical Species. The FASEB Journal, 32: 833.5-833.5.
- Jeon, J.Y., Ayyar, V.S. & Mitra, A. Pharmacokinetic and Pharmacodynamic Modeling of siRNA Therapeutics – a Minireview. Pharm Res 39, 1749–1759 (2022).
- Nair JK, H Attarwala , A Sehgal , Q Wang , K Aluri , X Zhang , M Gao , J Liu , R Indrakanti , et al. (2017). Impact of enhanced metabolic stability on pharmacokinetics and pharmacodynamics of GalNAc-siRNA conjugates. Nucleic Acids Res 45:10969–10977.
About the authors
- Dr. Nan Zhao heads the non-GLP bioanalytical in vivo screening team at WuXi AppTec in Shanghai and oversees the report team within the DMPK services department.
- Dr. Jiaming Jiang, a senior scientist at WuXi AppTec, specializes in the bioanalysis of nucleic acids.
- Zhiyu Li, a director in the DMPK services department at WuXi AppTec in China, oversees bioanalysis of large molecule biomarkers and laboratory testing.
- Lili Xing, a senior director in the DMPK services department at WuXi AppTec in China, leads the non-GLP bioanalysis team in the laboratory testing division.